The Antibacterial Mechanisms of Silver Nanoparticles
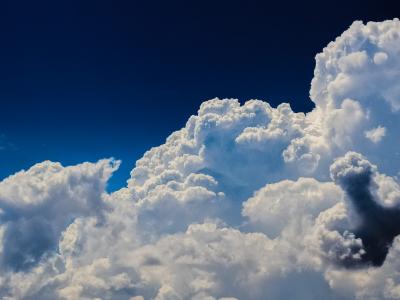
Abstract
The in-depth knowledge of antibiotic bacterial resistance mechanisms makes it possible to modify the chemical structures of existing antibacterial drugs so they regain their effectiveness.
Multidrug resistance reduces the risk-benefit ratio of antibiotics due to cumulative toxic effects of associated drugs. To antagonize bacterial resistance, it is attempted to introduce other therapeutic alternatives into the therapy alongside existing molecules. One of these is represented by silver nanoparticles (AgNp). Various technologies for obtaining AgNp are described in the literature, physical, chemical, biological and microbiological methods. Of these, microbiological methods offer important pharmacotherapeutic prospects, because it can optimize the antibacterial spectrum of nanoparticles.
The mechanisms of action of silver nanoparticles are still incompletely elucidated, but some subcellular and molecular targets are known, such as enzymes of energy metabolism, ribosomes and microbial DNA.
Improving the efficacy, stability, specificity, safety and biocompatibility of AgNp, administered alone or in combination with classical antibacterial drugs (antibiotics), can make them therapeutically viable alternatives to antimicrobial therapy.
Table of Contents:
1. Introduction
2. Safety [6]
3. Synthesis methods of silver nanoparticles
4. The antibacterial mechanisms of silver nanoparticles [16]
5. Conclusions
1. Introduction
European Union, through Scientific Committee on Emerging and Newly Identified Health Risks (SCENIHR), have defined nanoparticles as particles with at least one dimension on the order of 100 nm or less [1]. In the last decades it has been developed synthesis methods of metal nanoparticles as a result of their widespread use in various areas of activity like paints industry, electronics, cosmetics and medicines, for example. Silver nanoparticles (AgNPs), among other compounds, are fetching a higher interest because their antimicrobial activity [2].
Bacteria possess the ability to counteract the action of antibiotics. Since the discovery of penicillin in 1940, bacteria have been evolving ceaselessly, decreasing in this way the antibacterial effect of antibiotics. The mechanisms through which bacteria develop resistance to antibiotics aren’t well known, but still, a part of them have been described in the literature [3]. The misuse of antibiotics triggers these mechanisms, which involve firstly, variations in the genes structure of bacteria. The antimicrobial resistance is not only occurring to antibiotics which were used for a long time to treat infections, nowadays, the mechanisms involved in this process affects new drugs, also.
For diminishing the spread of multidrug resistance bacteria. it is necessary to discover new antimicrobial compounds, to modify the current structure of existing antibiotics and also to introduce new alternatives for antibiotic therapy, like AgNPs [4].
Silver had been used hundreds of years before the discovery of pathogenic agents. Hippocrates used silver for accelerating wounds healing. In the second-world war, water was preserved in silver recipients, same as in the periode of Persian kings. In the sixteen centuries, Paracelsus used silver nitrate by oral route and also cutaneous for treat wounds. Later, in 1852, physician J. Marion Sims used for the first-time silver sutures in surgical procedures. Nowadays, silver si used as AgNPs, frequently in burns treatment [5], and it’s becoming a viable option for treating infections caused by multidrug resistance bacteria.
2. Safety [6]
Evan if nanotechnology field is growing fast, there is a lack of information regarding human exposures to AgNPs. In vitro tests on a mouse spermatogonial stem cell line showed that AgNPs can reduce mitochondrial function and it can increase membrane leakage [7]. The acute toxic effects of AgNPs was assessed by Hussain et al, using the in vitro rat-liver-derived cell line (BRL 3A). It was observed a Lactate dehydrogenase (LDH) leakage for the cells exposed to 10-50μg ml−1 AgNPs [8].
The depletion of reduced glutathione (GSH), beside the reduced mitochondrial membrane potential and the increase in reactive oxygen species (ROS) levels, suggest that cytotoxicity of AgNPs in liver cells is mediated through oxidative stress. On human fibrosarcoma and human carcinoma cells in vitro, concentration levels of AgNPs ranged from 1.56 to 6.25 μg ml−1 were reported safe [9].
The mechanism of AgNPs mediated cytotoxicity is generally based on induction of ROS, reduction of GSH levels, DNA damage, apoptosis and necrosis. The cytotoxicity level depends on particle size, concentration and exposure time. For assessing the in vitro cytotoxicity and genotoxicity where made important advances comparably to evaluating in vivo toxicity for mammals and humans.
Due to the ultra-little sizes of Ag-NPs, an extraordinary versatility is given in various conditions. Humans can be exposed via oral route, ingestion or inhalation. The AgNPs can easily translocate
into another region of the body, to another organ, different from the exposure route.
For example, Ji et al., worked in a study on Sprague-Dawley rats for 28 days to evaluate the inhalation toxicity induced by AgNPs. Surprisingly, they found no harm was produced by inhaled AgNPs [10].
Another study, made by Kim et al., on the same species of rats, showed that exposure to doses higher than 300 mg of AgNPs for a period of at least 28 days, modifies values for the alkaline phosphatase and cholesterol, producing in this way liver damage [11].
Altough argyria disappeared after silver was eliminated from oral drugs, the current practice of health treatments using colloidal silver get in focus again this disease. Chang A. et al., have reported a case of argyria in 2006. The skin biopsy revealed that colloidal silver was the source of the patient dyspigmentation [12].
3. Synthesis methods of silver nanoparticles
Synthesis methods of silver nanoparticles are divided in four categories: physical, chemical, photochemical and biological methods. Physical and photochemical methods are ussualy expensive.
These methods involve high temperatures and vacuum conditions. Using chemical methods, it results pure and fine-shaped nanoparticles, with a high yeld. The equipment used for synthetize AgNPs is simple and costless comparing to physical and photochemical ones. By adjusting the reaction parameters, it can be obtained AgNPs with different shapes and dimensions [13]. Both chemical and physical methods are generally hazardous. The reducing agents used in chemical methods are very harmful to living organisms. Biological synthesis approach is more often used, replacing the other methods. Beeing simple, environmentally friendly, cost effective and dependable, biological synthesis methods represent a viable option. Bacteria, fungi, plant extracts biopolymers, starch, fibrinolytic enzyme and aminoacids were used to synthetize AgNPs.
- Bacteria: Pseudomonas stutzeri AG259, Lactobacillus strains, Bacillus licheniformis;
Escherichia coli, Brevibacterium casei;
- Fungi: Fusarium oxysporum, Ganoderma neo-japonicum Imazeki;
- Plant extracts: Allophylus cobbe, Artemisia princeps, Typha angustifolia [14].
Some bacteria possess natural resistance to AgNPs. It is the case for Pseudomonas stutzeri AG259, a silver-resistant bacterial strain isolated first time from a silver mine. In a study, during culturing, in the presence of high concentrations of silver salt, the bacterial cell accumulated silver and formed intracellular AgNPs with sizes raging from a few nm to 200 nm and different shapes [15].
4. The antibacterial mechanisms of silver nanoparticles [16]
The antimicrobial potential of AgNPs is influenced by their colloidal state, size, shape and also by the surface charge, and concentration. AgNPs have antibacterial, antifungal and antiviral action and are considered the next class of antibiotics. In the literature are described many mechanisms of action, not fully elucidated but only four of them are in depth known.
1. Adhesion of AgNPs onto the surface of cell wall and membrane
2. AgNPs penetration inside the cell and destabilization of intracellular structures and biomolecules
3. AgNPs induced cellular toxicity and oxidative stress
4. Modulation of signal transduction pathways
1. Adhesion of AgNPs onto the surface of cell wall and membrane
AgNPs adhere on to the cell wall and the membrane. The positive charge confers electrostatic attraction between AgNPs and negatively charged cell membrane of the microorganisms, thereby facilitates AgNPs attachment onto cell membranes. Morphological changes become evident upon such interaction and can be characterized by shrinkage of the cytoplasm and membrane detachment, finally leading to rupture of cell wall.
2. AgNPs penetration inside the cell and destabilization of intracellular structures and biomolecules AgNPs can penetrate inside the microbial cell, it may interact with cellular structures and biomolecules such as proteins, lipids, and DNA, resulting damaging effects on microbes. In particular, AgNPs interaction with ribosomes leads to their denaturation causing inhibition of
translation and protein synthesis.
3. AgNPs induced cellular toxicity and oxidative stress
Increase in cellular oxidative stress in microbes is a sign of toxic effects caused by heavy metals ions, such as silver ions. Therefore, increased concentration of silver ions is expected to cause an increase in cellular oxidative stress. The potent antibacterial, antifungal and antiviral activity of AgNPs is due to their ability of producing ROS and free radical species such as hydrogen peroxide (H2O2), superoxide anion (O2−), hydroxylradical (OH•), hypochlorous acid (HOCl) and singlet oxygen.
4. Modulation of signal transduction pathways
Phosphorylation of various protein substrates in bacteria is widely recognised. Examining the phosphotyrosine profile of bacterial proteins from both Gram-positive and Gram-negative offers a useful way to study the effect of AgNPs on bacterial signal transduction pathways. These signaling pathways affect bacterial growth and other molecular and cellular activities. The reversible phosphorylation of tyrosine residue of protein substrates, such as RNA polymerase sigma factor, single-stranded DNA binding proteins and UDP glucose dehydrogenase, lead to their activation.
The resultant phosphorylated proteins have essential role in DNA replication, recombination, metabolism and bacterial cell cycle. Therefore, inhibition of proteins phosphorylation would inhibit their enzymatic activity, which in turn will result in inhibition of bacterial growth. AgNPs probably modulates cellular signaling and acts by dephosphorylating tyrosine residues on key bacterial peptide substrates and thus inhibit microbial growth.
5. Conclusions
The fact that pathogens are increasingly aggressive, managing to adapt to new antimicrobial therapies by acquiring multidrug resistance, is a challenge to improve existing molecules to increase the efficiency and success of treatment [17-19]. In this regard, antibacterial action of silver nanoparticles offers pharmacotherapeutic prospects, but requires an exhaustive mechanistic approach.
The authors:
BLEJAN Emilian Ionuț [1]
ARSENE Andreea Letiția [1]
[1] “Carol Davila” Univerity of Medicine and Pharmacy, Faculty of Pharmacy, Department of General and Pharmaceutical Microbiology (ROMANIA).
Contributo selezionato da Filodiritto tra quelli pubblicati nei Proceedings “17th Romanian National Congress oh Pharmacy – 21st Century Pharmacy – Between Intelligent Specialization and Social Responsibility - 2018”
Per acquistare i Proceedings clicca qui.
Contribution selected by Filodiritto among those published in the Proceedings “17th Romanian National Congress oh Pharmacy – 21st Century Pharmacy – Between Intelligent Specialization and Social Responsibility - 2018”
To buy the Proceedings click here.